Electrolysis of seawater
Published on 4 June 2022
Water electrolysis to produce hydrogen requires electricity and water as main inputs. Because existing electrolysers run on purified water, the most common approach is to use fresh water that is further purified.
However, nonfrozen fresh water represents less than 1% of global water resources and is very heterogeneously distributed on the planet. Furthermore, a large fraction of renewable energy potential is offshore, i.e. in or just above seawater. Accordingly, there is an interest in using seawater as base resource for green hydrogen production.
Water composition and electrolyser requirements
Fresh water and seawater are distinguished by their content in dissolved salts. Fresh water contains less than 500 ppm of dissolved salts. Sea water contains on average about 35,000 ppm of dissolved salts, with significant variations from places to places.
None of these resources are directly compatible with existing electrolysers that require pure water, defined as having a content of less than 10 ppm of dissolved salts. For this reason, any industrial electrolyser includes a purification stage.
Seawater has a variable composition but a representative average composition can be defined as approximately containing 21,000 ppm of chlore, 12,000 ppm of sodium, 2000 ppm of sulphur, 1300 ppm of magnesium, 400 ppm of calcium and potassium, 70 ppm of brome, 30 ppm of carbon and 10 ppm of nitrogen. In addition to these salts, there is a significant content in microorganisms as well as a pollution-based content like plastic particles, heavy metal traces, ...
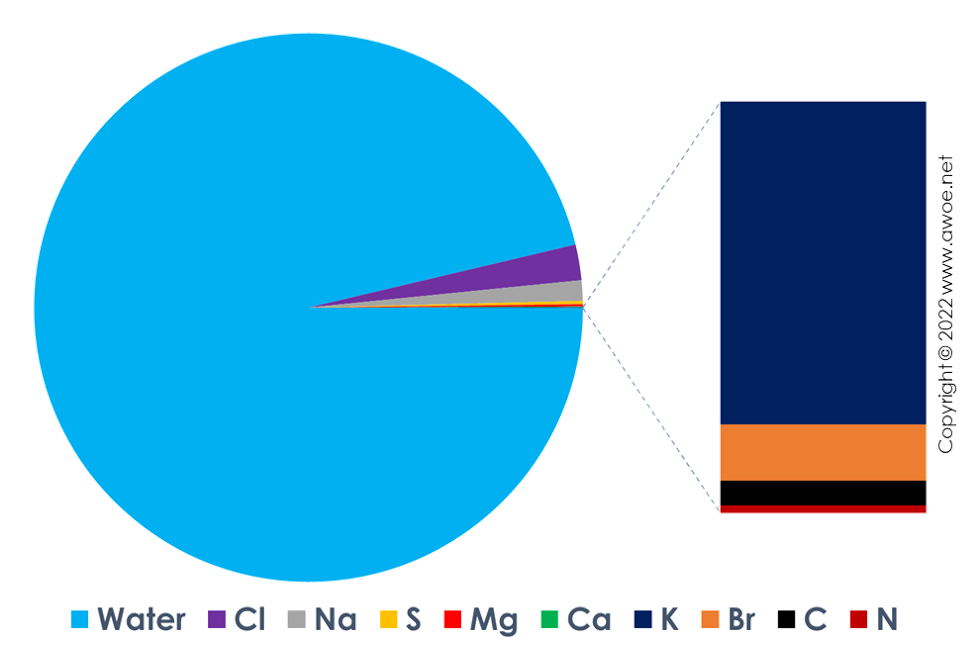
Average seawater composition
Given the large content in salts, using seawater for electrolysis could be done either by adapting the electrolysers to seawater or by desalination of seawater with a similar process to fresh water production for human consumption.
Electrolyser adaptation
Directly using seawater would require building an electrolyser that can accept large salt content as an input and includes a method to deal with microorganisms. The seawater desalination industry shows that absorbing microorganism creates fouling on the long term, so that pre-filtering or treatment with UV light is a likely requirement.
Salts within seawater can be classified into 3 categories:
- Salts that interact with the electrochemical activity, mainly chlore and brome
- Salts that create large amounts of deposits, mainly sodium and magnesium
- Others that have minor effects and therefore do not require much additional treatment
Chlore and brome salts compete with the oxygen production reaction at the anode because their electrochemical reactions operate around the same potential and their kinetics is quicker than that of water molecule splitting. Within an acidic environment (PEM electrolysers), the overpotentials are so similar that very advanced electrode materials are required to favour the oxygen production reaction, but today these are not durable. Within an alkaline environment (alkaline electrolysers), the overpotential difference is larger and more conventional compounds as well as simpler production methods still ensure a good selectivity in favour of water splitting.
Sodium and magnesium accumulate as deposits in the electrolyser cells, especially in alkaline environments (pH > 8.5), if not separated beforehand. The simplest identified solution is to pre-treat seawater by adding an alkali in a buffer tank to favour deposition where deposits can be collected and extracted.
While such individual adaptations have already been independently demonstrated in laboratories, there exists yet no industrial electrolyser combining all adaptations.
Seawater pre-treatment
Although technology is progressing to be able to directly use seawater in electrolysers (realistically only in alkaline electrolysers given the cost of specific adaptation for PEM), the economics might push towards using a two-step process of water purification and electrolysis of purified water.
Reverse osmosis is a well-established technology to convert seawater (35,000 ppm of salts) into fresh water (< 500 ppm of salts). Research has already demonstrated that flowing such fresh water a second time into the reverse osmosis equipment can yield purified water with less than 5 ppm of salts, making it compatible with electrolysers requirements. Besides, the energy consumption of the second pass of purification is minor.
Given the relatively low water consumption of electrolysers to convert electric energy into chemical energy stored in hydrogen, existing reverse osmosis units could easily deal with such demand. Furthermore, the energy consumption to run the reverse osmosis process is of the order of a thousandth of the electric consumption of electrolysis, both treating the same amount of water.
Accordingly, a two-step process of seawater purification and conventional electrolysis has the advantage of only relying on existing technology while not adding massive investment or running costs.
Demonstrator with seawater pre-treatment
A world-first two-step installation is currently developed by Vattenfall in Aberdeen, Scotland. The Hydrogen Turbine 1 (HT1) project has been recently granted £9.3 million by the UK government to update an offshore wind turbine platform to host a hydrogen production unit as well as to install a subsea hydrogen pipeline and an onshore hydrogen storage unit. The Screening Opinion Request Report describes adding seven 40ft containers to an 8.8 MW offshore turbine platform for:
- Seawater pumping (including pumps, filters and buffer tank, up to 3.52 m3/h)
- Desalination (rejection of brine ~50% more concentrated in salts than pumped water, up to 1.76 m3/h)
- Di-ionisation (converting desalinated water into pure water)
- PEM electrolyser for hydrogen production (up to 0.18 m3/h)
- Compressor for hydrogen distribution (up to 200 bar)
- Onshore hydrogen storage and tube trailer refueller compatible with production of up to 4 tons of hydrogen per day
With an electrolyser directly installed on the wind turbine platform, a large variability in electricity output can be expected, hence the choice of a PEM electrolyser. Beyond feasibility demonstration (operational in 2024/2025), the main publicised goal of the project is to establish the real-life efficiency of such an “island” installation (no connection to grid, no support from any other power source).
Perspectives
Conversion of alkaline electrolysers to work on seawater seems technologically feasible, while such a conversion is more challenging for PEM electrolysers. However, offshore or coastline electrolysis installations would interact with intrinsically variable electricity sources, something that lends itself better to direct coupling with PEM electrolysers than alkaline electrolysers.
Alternatively, seawater electrolysis could simply combine existing technologies, namely any desalination method and any electrolyser without much added costs. Indeed, total cost is the combination of running cost (mainly electricity here) and capital cost, with desalination being largely cheaper than electrolysis in both categories.
Green hydrogen production from seawater based on electricity harvested in marine environment is therefore not an impossible technological feat, although not common today because the few existing electrolysis plants are installed where fresh water is available. Results from Vattenfall demonstrator in Scotland might change perception and open a new path towards a large hydrogen economy.